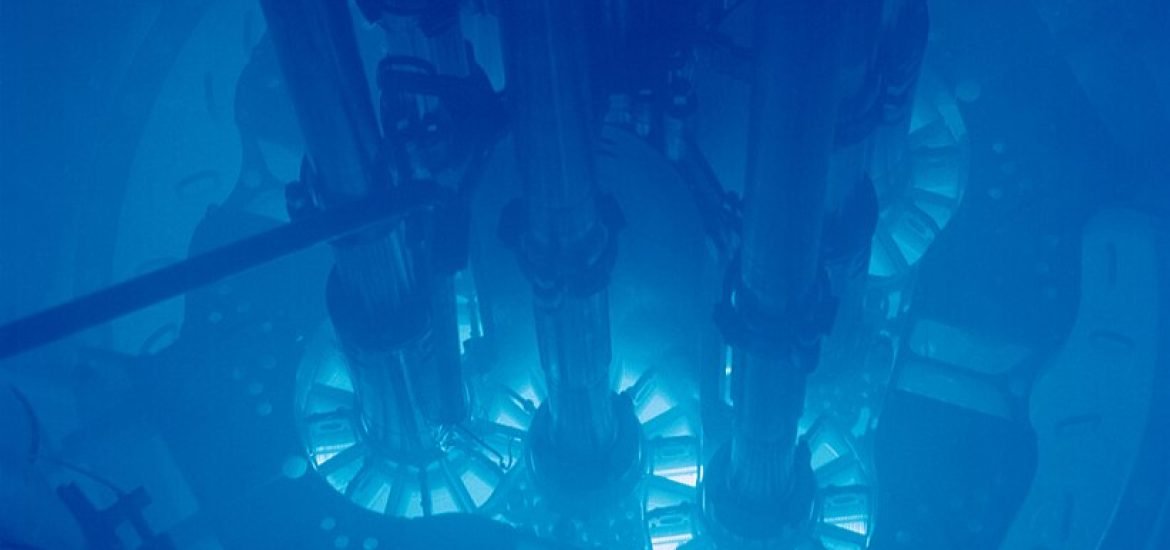
Delve into the second installment of ‘Nuclear Power Takes Off,’ penned by Samuel Furfari, esteemed Professor at the University of Brussels, and Ernest Mund, distinguished Emeritus Extraordinary Professor at UCL. Building upon the groundwork laid in the initial segment which highlighted the escalating demand for electrical power, this next chapter unfurls the captivating narrative of nuclear technology’s historical evolution.
The present lock-in of nuclear power
On December 2, 1942, a team of physicists led by Enrico Fermi at the University of Chicago performs the first neutron chain reaction in a graphite-moderated pile called CP-1. This chain reaction is based on nuclear fission of uranium-235, a physical phenomenon discovered in Germany four years earlier. A new technology emerges with physical processes releasing extremely large amounts of thermal energy. At that time the United States, the top priority is a rapid end of World War II. Obviously, the new technology has many military applications. These are immediately developed in the frame of the so-called Manhattan Project leading to the destruction of Hiroshima and Nagasaki in 1945. After the end of WWII, a new threat emerges that also asks for military strategy: Soviet imperialism. At that time some high commanders of the US Navy envisage nuclear propulsion for submarines as a main deterrent, allowing long-term underwater immersion. Similar prospects arise at the US Air Force, although, from the start, they look more difficult to achieve.
Early on physicists were aware of the variety of possible chain-reacting systems depending on the choice of moderator (a light element such as hydrogen, deuterium or carbon for the slowing down of high energy fission neutrons) and coolant (light – or heavy water, gas or liquid metals). The graphite-moderated CP-1 reactor offered an excellent neutron balance but graphite leads to less compact facilities than water-moderated ones. Light water reactors (LWR) on the other hand have a less favourable neutron economy than graphite – or heavy water (D2O) moderated reactors. But reactor size being imperative for submarine propulsion systems, LWR were chosen for the US Navy (Weinberg, 1994). As industry giants like Westinghouse and General Electric were very soon involved in the naval programme, the commercial power reactors that would follow would naturally belong to the same technology, requiring slightly enriched uranium in U235 ≤5%). The United Kingdom and France initially opted for natural uranium (Unat) and graphite, while Canada chose Unat and heavy water for its CANDU line. Over time, only CANDU reactors among the varieties based on Unat were maintained, the English and French graphite-moderated and gas-cooled reactors being gradually supplanted by the suboptimal LWRs, enforcing lock-in (Cowan, 1998).
Shippingport, the first civilian PWR reactor (Generation-II) was launched in 1957 with a 60 MWe power output. Five years later came BR3, the first PWR installation outside the US, built at SCK•CEN Mol in Belgium. Shippingport was a landmark in many respects. In particular, it demonstrated the possibility to breed (1) U233 from Th232 with thermal neutrons. Thermal neutron breeding though it was not exploited, R&D being focused at the time essentially on fast neutron breeding.
First LWR commercial units built in the early ’70s were rated well above 400 MWe to optimise the investments and reduce energy costs. A basic feature of the starting industry – with obvious economic consequences – was that no two power plants were exactly the same. This practice hasn’t changed, although technical elements are progressively put in place that could modify LWR concepts within the next decade. Advances in LWR technology at Oregon State University (OSU) Portland during the ’90s, with the development of so-called integral PWRs, having their steam generators (SG) inside their pressure vessels, reducing considerably the risk of loss-of-coolant accident (LOCA), have been the start of a new industrial paradigm: factory-made units of much smaller power size ∼75 MWe), the so-called Small Modular Reactor (SMR) concept. Figure 6 illustrates the difference between conventional and SMR designs of LWRs. Note the inclusion of the SG inside the reactor vessel with a shortened primary coolant circuit in the latter design. NuScale, an offspring of the OSU nuclear R&D team, will likely be the first SMR designer to install a power plant comprising six 77 MWe power modules at the Idaho National Laboratory (INL) before the end of the decade. Other light-water SMR systems with integrated SG will soon appear on the market in China (ACP100), France (Nuward) and the UK. In the latter case Rolls-Royce – better known for its luxury cars – is the architect-engineer of British nuclear submarines developing a 470 MWe pressurised water SMR. In March 2023, it signed a Memorandum of Intent with the Polish group Industria to deploy three SMRs to decarbonise the company energy infrastructure.
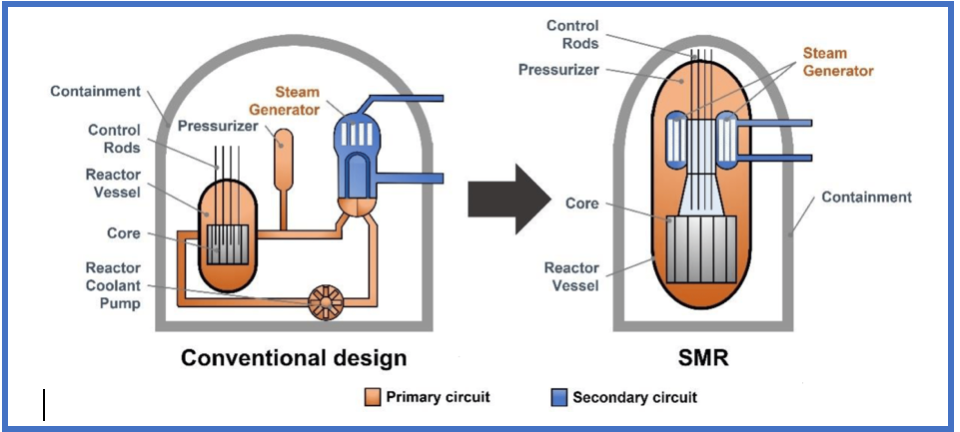
The economic competitiveness of SMRs versus large reactors is a key topic. It has been extensively studied during the last decade (Boarin et al., 2012) and still is (Mignacca and Locatelli, 2020) no definite conclusion having emerged. Most authors agree that the scale effect isn’t the only factor to be considered, co-siting economies, modularity, and construction time being quite relevant parameters as well. Finally, there is an agreement on the need for an SMR-site decommissioning cost analysis to be compared with the decommissioning cost of large power plants. Decommissioning multiple smaller installations could be less costly but needs assessment.
Early activities in advance reactor systems
At the time the US Navy endorsed nuclear propulsion for its submarines, the US Air Force was looking for aircraft nuclear propulsion (ANP). Water reactors being unable to provide temperature levels needed for aircraft propulsion ∼750 °C), physicists at Oak Ridge National Laboratory (ORNL) suggested an alternate neutron chain-reacting system based on molten salts providing high temperatures. The first system known as ARE (Aircraft Reactor Experiment) went critical in 1954. It was followed by MSRE (Molten Salt Reactor Experiment) in 1965 which operated for four years. Both systems were very successful. The technology differed fundamentally from Fermi’s original one, with a uranium tetrafluoride fluid fuel instead of a uranium metallic one. At that time, Alvin Weinberg and Eugene Wigner were fascinated by homogeneous nuclear systems, easy to model mathematically. They promoted the development of ORNL of two systems using a highly enriched uranium oxide sulphate aqueous solution: the Homogeneous Reactor Experiment (HRE) and Homogeneous Reactor Test (HRT). These four successful facilities – ARE, MSRE, HRE, HRT – are perhaps the most important ones among the 13 that were built at ORNL, about the design of nuclear systems for the future, dubbed Generation-IV (Rosenthal, 2010).
Nuclear energy of the future
In 2001, the US Department of Energy (USDOE) launched an initiative to identify nuclear technologies that meet stringent safety, environmental, economic and non-proliferation criteria for future primary energy production. A study group called Generation-IV International Forum (GIF) was created. Several countries responded to the call (2) and identified six technologies that met these requirements: gas cooled fast reactors (GFR), sodium-cooled fast reactors (SFR) supercritical water reactors (SCWR) very high temperature reactors (VHTR), lead cooled fast reactors (LFR) and molten salt reactors (MSR), symbolically represented in Figure 7. The molten salt technology that had been tested at ORNL during the 1950s in the ARE and MSRE experiments was considered by the GIF experts as one of the most attractive and safe nuclear technologies for the following reasons:
- The absence of water in the reactor core eliminates the risk of a loss-of-coolant accident (LOCA), as was the case in the Three Mile Island accident in 1979. It also eliminates the risk of hydrogen explosion from zirconium/water reactions that can occur on exposed high-temperature fuel rods cladding (an event that occurred at Fukushima);
- The absence of sodium eliminates the risk of highly exothermic chemical reactions;
- A pressure close to atmospheric pressure, the liquid state of the fuel and favourable neutron properties (strongly negative temperature reactivity coefficient) also contribute to safety;
- The fact that operating temperatures ∼700 °C) the fuel for MSRs is in the liquid phase makes it easier to feed fresh fuel than in solid fuel systems. Although this is a delicate operation, a regular supply is possible, which is not the case for solid fuel systems. There is therefore no need for a long-term reactivity reserve, which eliminates the risk of a Chernobyl-type criticality accident;
- The composition of the molten salt is such that the liquid state can be maintained up to 1,400 °C, well above operating values. If for any reason the temperature of the salt falls below 459 °C, the melting temperature of the lithium beryllium fluoride (LBF) of which it is composed, solidification occurs with mass retention of the non-volatile fission products.
All of these were outlined in detail in the first Generation-IV technology roadmap (U.S. DOE NERAC, 2002).
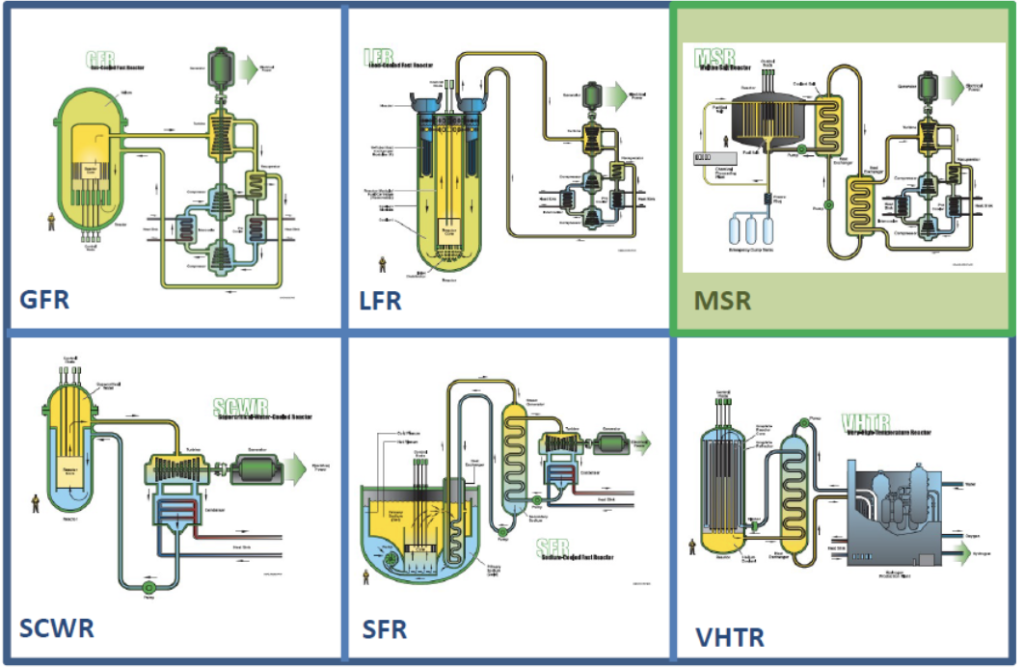
MSR’s reduced accident risks have a positive impact on the reactor economy, by reducing the need for active systems to guarantee the safety of the installations, such as those used in current LWR plants. Finally, the ultimate interest: molten salt installations with fast neutron spectra would open the way to a reduction in the nuclear ‘liabilities’ of current reactors (plutonium and minor actinides with very long half-lives) that are unmanageable in the latter. Inserting this spent fuel as a fuel source in these SRMs would simultaneously exploit its residual energy content and eliminate a highly problematic environmental presence of radioisotopes with several hundred thousand half-lives.
The conclusions of the Generation-IV Roadmap gave rise throughout the world to a revival of interest in technology that had entered lethargy in the early 1980s. Many current MSR R&D projects should lead to prototypes within the next 15 to 20 years (Furfari and Mund, 2022, IAEA 2020). Figure 8 displays some of the most advanced ones in various countries.
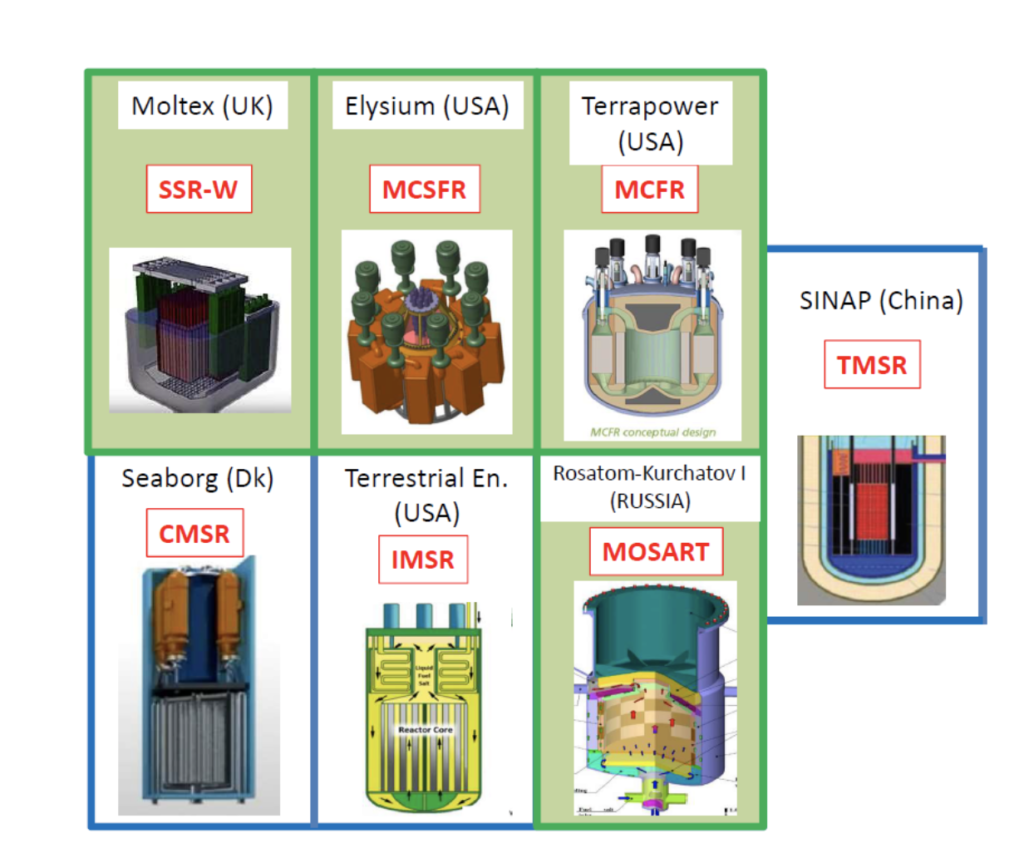
Attention should be paid to the Moltex, Elysium, TerraPower and Mosart projects. These are four fast reactor projects specifically designed to exploit not only conventional resources (235U and 239Pu produced from the conversion of 238 U) but also the existing stock of spent fuel from current LWR and CANDU reactors with a significant range of actinide nuclei. While doing this, they transform an environmentally unsustainable easement – for the most ardent opponents of nuclear power – into an energy resource that can be used until all contained fissile nuclei are exhausted. TMSR, as its name indicates, is oriented towards the exploitation of the thorium fuel cycle, an important mineral asset in China. TMSR is the only facility developed along with two different options: a molten salt fuel version or a solid fuel version using pebbles with TRISO particles (Dalin Zhang et al., 2018).
Most installations enter the SMR category, with electric power units less or equal to 300 MWe. Depending on the intended applications, power units may be as low as 45 MWe. This is the case for instances of Terrapower’s MCFR that has been selected by Core Power, a British maritime company to decarbonise naval propulsion (Furfari and Mund, 2022).
Future MSR systems will coexist with Generation-II and Generation-III LWRs with power sizes exceeding 1.6 GWe. Their nuclear fuel cycles will be in synergy as illustrated by Figure 9. Basically, all will depend on uranium mining, but MSRs with fast neutron spectra will eliminate minor actinides generated in LWRs and reduce the long-lived nuclear waste stockpile. All facilities will provide electricity but in addition MSR will also deliver high-temperature heat for industrial uses (district heating, water desalination) and final energy for naval propulsion.
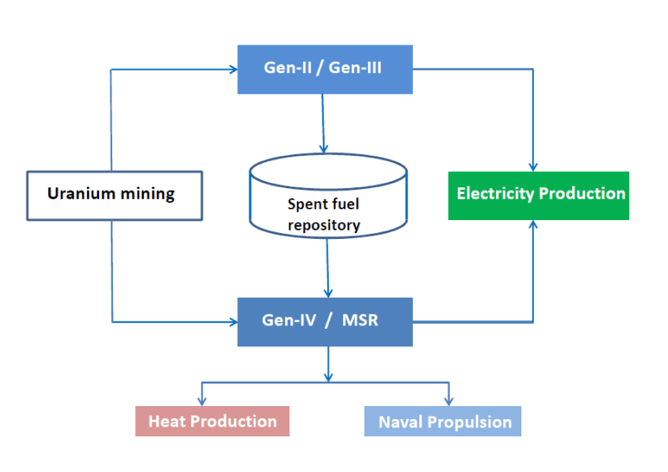
Industrial heat production is of the utmost importance as outlined in (Furfari and Mund, 2020). In current energy policies, the use of heat is too often neglected. The 2022 crisis in Germany has highlighted this fundamental need for ‘final energy’, which the chemical industry badly needs. Ninety-four percent of the natural gas used in Germany is used for thermal purposes.
The MSR heat may also become an energy source for the thermal decomposition of water and hydrogen production. An EU policy Green Deal requirement for accelerated hydrogen production from variable and intermittent renewables may lock in this hydrogen production despite its economic and environmental disadvantages shown below.
End of part 2/3
Read part one : Nuclear power takes off (Part 1 ): meeting the growth in global electricity demand
Read part three : Nuclear power takes off (Part 3) : Back to Euratom ?
(1) i.e. produce fissile nuclei in excess of nuclei consumption entailed by energy production.
(2) Argentina, Brazil, Canada, France, Japan, South Korea, South Africa, the United Kingdom and the United States, later joined by Switzerland, the EU, China, Russia and Australia
(3) The acronyms read as follows : SSR-W (Stable Salt Reactor Wasteburner), MCSFR (Molten Chloride Salt Fast Reactor), MCFR (Molten Chloride Fast Reactor), CMSR (Compact Molten Salt Reactor) and IMSR (Integral Molten Salt Reactor), TMSR (Thorium Molten Salt Reactor).
References
Boarin S., Locatelli G., Mancini M, & Ricotti M.E. (2012). Financial Case Studies on Small- and Medium-Size Modular Reactors. Nuclear Technology, volume 178, 218–232.
Cowan R. (1990). Nuclear Power Reactors: A Study in Technological Lock-in. The Journal of Economic History, volume 50, 541–567.
Dalin Zhang & al. (2018). Review of conceptual design and fundamental research of molten salt reactors in China. Int J Energy Res. 2018; 42:1834-1848,
Furfari S., & Mund E. (2020), Renewable energy in the EU: from perception to reality, Energy Literacy, 27-11-2020, https://www.connaissancedesenergies.org/tribune-actualite-energies/energies-renouvelables-dans-lue-de-la-perception-aux-realites.
Furfari S., & Mund E. (2022). Advanced nuclear power for clean maritime propulsion, Eur. Phys. J. Plus 137:747.
Mignacca B., & Locatelli G. (2020). Economics and finance of Small Modular Reactors: A systematic review and research agenda. Renewable and Sustainable Energy Reviews 118.
Rosenthal, M. W. (2010). An Account of Oak Ridge National Laboratory’s Thirteen Nuclear Reactors. ORNL/TM-2009/181.
Weinberg A.M. (1994). The First Nuclear Era: The Life and Times of a Technological Fixer. Springer New York.
U.S. DOE NERAC. (2002) A Technology Roadmap for Generation IV Nuclear Energy Systems https://www.gen-4.org/gif/upload/docs/application/pdf/2014-03/gif-tru2014.pdf.
Par Argonne National Laboratory — originally posted to Flickr as Advanced Test Reactor core, Idaho National LaboratoryUploaded using F2ComButton, CC BY-SA 2.0, https://commons.wikimedia.org/w/index.php?curid=27024528